In light of the recent exciting press release about the supermassive black hole at the center of our own Milky Way galaxy, I am going to discuss a brief introduction to black holes and other related concepts in a series of blog articles for those who wish to understand better these amazing monstrous heavenly creatures. For starter, this first article is about Schwarzschild black hole, the simplest black hole model and also the first exact solution of the Einstein’s Field Equation.
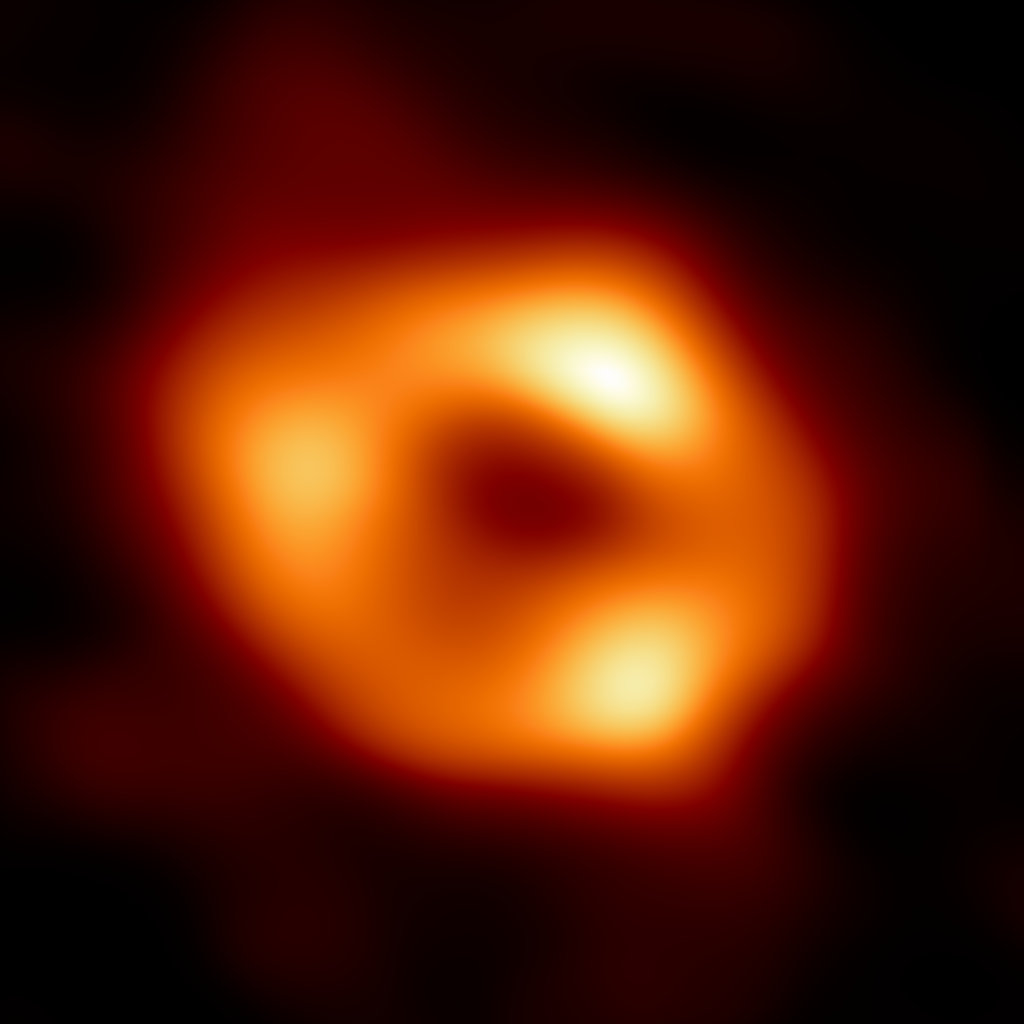
First, what is a black hole?
A black hole is a hypothetical (though its existence is no longer in doubt) region of spacetime where gravity is so immense that nothing, even light (the lightest matter in the universe) can escape from it.
How did we come to know about a black hole? Was it just an imagination of some mad scientists?
No imagination. We came to know about the theoretical notion of a black hole from Einstein’s general relativity. But long before general relativity came out, French mathematician Pierre-Simon Laplace had already thought about a celestial object whose gravity is so strong that even light can’t escape. It is discussed in his five-volume book Mécanique Céleste (Celestial Mechanics).
In general relativity, black holes are solutions of the Einstein’s Field Equations.
What are the Einstein’s Field Equations?
They are the fundamental equations of Einstein’s theory of gravitation. Such equations are expected to satisfy the following requirements:
- The field equations should be independent of coordinate systems, i.e. they should be tensorial.
- They should be partial differential equations of second order for the components $g_{ij}$ of the unknown metric tensor. If you want to know what a metric tensor is, go deeper here.
- They are a relativistic generalization of the Poisson equation of Newtonian gravitational potential $$\nabla^2\Phi=4\pi G\rho$$ where $\rho$ is the mass density.
- Since the energy-momentum tensor $T_{ij}$ is the special relativistic analogue of the mass density, it should be the source of the gravitational field.
- If the space is flat, $T_{ij}$ should vanish.
The equations satisfying all these requirements are given by \begin{equation}\label{eq:EFT}G_{ij}=8\pi GT_{ij}\end{equation} where $G_{ij}=R_{ij}-\frac{1}{2}Rg_{ij}$. $G_{ij}$ is called the Einstein tensor. $R_{ij}$ and $R$ are, respectively, Ricci curvature tensor and scalar curvature. If you want to know what they are, go deeper here. Interestingly, the field equations \eqref{eq:EFT} were derived independently and almost simultaneously by Albert Einstein and David Hilbert in 1915.
So, how do we solve the Field Equations?
The field equations are nonlinear partial differential equations. Partial differential equations are, in general, difficult to solve with equations alone. We impose additional (physical) assumptions and conditions (such as boundary conditions and initial conditions) so the equations can becomes simpler enough for us to be able to solve. First, we have no information on the source of gravity, i.e. no information on the energy-momentum tensor $T_{ij}$. Instead, we consider the field equations outside the field-producing masses. Thus, we obtain the vacuum field equations $$R_{ij}-\frac{1}{2}Rg_{ij}=0$$ Without loss of generality, we may assume that the metric tensor $g_{ij}$ is diagonal, i.e. $g_{ij}=0$ if $i\ne j$. This results in $R_{ij}=0$ if $i\ne j$ from the vacuum field equations. For $i=j$, since the scalar curvature $R$ is given by $R=\sum_ig^{ii}R_{ii}$, from the vacuum field equations, we have $(n-2)R=0$. For $n=4$, $R=0$ and therefore the vacuum field equations turn into \begin{equation}\label{eq:ricciflat}R_{ij}=0\end{equation} i.e. vanishing Ricci curvature tensor. \eqref{eq:ricciflat} is simpler than the original vacuum field equations but by no means it is easy to solve. The simplest solution of \eqref{eq:ricciflat} was obtained in 1915 by a German physicist Karl Schwarzschild while serving in the army during World War I. This is also the first exact solution to the Einstein’s field equations. Schwarzschild assumed a static spherical symmetric metric as a solution of the vacuum equations \eqref{eq:ricciflat}. The ansatz for a static isotropic metric is given by \begin{equation}\label{eq:ansatz}ds^2=-A(r)dt^2+B(r)dr^2+r^2(d\theta^2+\sin^2\theta d\phi^2)\end{equation} In addition, we want the solution to become the flat spacetime far away from the masses i.e. the solution is asymptotically flat, so we require $$\lim_{r\to\infty}A(r)=\lim_{r\to\infty}B(r)=1$$ Now, we are ready to solve the vacuum field equations \eqref{eq:ricciflat}. From the ansatz \eqref{eq:ansatz}, we have $$g_{tt}=-A(r),\ g_{rr}=B(r),\ g_{\theta\theta}=r^2,\ g_{\phi\phi}=r^2\sin^2\theta$$ The nonzero Christoffel symbols are computed to be \begin{align*}\Gamma_{rr}^r&=\frac{B’}{2B},\ \Gamma_{tt}^r=\frac{A’}{2B},\ \Gamma_{\theta\theta}^r=-\frac{r}{B}\\\Gamma_{\phi\phi}^r&=-\frac{r\sin^2\theta}{B},\ \Gamma_{\theta r}^\theta=\Gamma_{\phi r}^\phi=\frac{1}{r},\\\Gamma_{tr}^t&=\frac{A’}{2A},\ \Gamma_{\phi\phi}^\theta=-\sin\theta\cos\theta,\ \Gamma_{\phi\theta}^\phi=\cot\theta\end{align*} Here, ‘ stands for $\frac{d}{dr}$. Using these Christoffel symbols, the Ricci tensors are computed to be \begin{align*}R_{tt}&=\frac{A^{\prime\prime}}{2B}-\frac{A’}{4B}\left(\frac{A’}{A}+\frac{B’}{B}\right)+\frac{A’}{rB}\\R_{rr}&=-\frac{A^{\prime\prime}}{2A}+\frac{A’}{4A}\left(\frac{A’}{A}+\frac{B’}{B}\right)+\frac{B’}{rB}\\R_{\theta\theta}&=1-\frac{1}{B}-\frac{r}{2B}\left(\frac{A’}{A}-\frac{B’}{B}\right)\\R_{\phi\phi}&=\sin^2\theta R_{\theta\theta}\end{align*} From the first two equations of the Ricci tensors above and by requirung them to be 0, $$BR_{tt}+AR_{rr}=\frac{1}{rB}(A’B+AB’)=\frac{1}{rB}(AB)’=0$$ So, we have $A(r)B(r)$=constant. Asymptotic flatness implies that $A(r)B(r)=1$. $R_{\theta\theta}=0$ along with $B(r)=\frac{1}{A(r)}$ and $A’B+AB’=0$ results in $A+rA’=(rA)’=1$. Hence, $A(r)=1+\frac{C}{r}$, where $C$ is a constant. By weak field approximation (Newtonian limit) , we obtain \begin{align*}A(r)=-g_{00}&=1+\frac{2\Phi}{c^2}\\&=1-\frac{2MG}{c^2r}\end{align*} where $\Phi=-\frac{GM}{r}$. This determines the constant $C=-\frac{2MG}{c^2r}$. Finally, the Schwarzschild solution is given by \begin{equation}\label{eq:bh}ds^2=-\left(1-\frac{2MG}{c^2r}\right)c^2dt^2+\left(1-\frac{2MG}{c^2r}\right)^{-1}dr^2+r^2(d\theta^2+\sin^2\theta d\phi^2)\end{equation} As I mentioned in the beginning, this is the simplest black hole model and also is the first exact solution of the Einstein’s Field Equations. It turns out that Schwarzschild solution is the only spherically symmetric solution to the vacuum Einstein equation \eqref{eq:ricciflat} due to
Birkhoff’s Theorem
In 1923, George David Birkhoff proved:
Theorem. Any spherically symmetric solution of the vacuum Einstein’s field equations \eqref{eq:ricciflat} must be static and asymptotically flat.
The Shape of a Black Hole
Is the converse of Birkhoff’s theorem true? Namely, is any static and asymptotically flat solution of the vacuum field equations necessarily spherically symmetric? I don’t know the answer, though I assume the answer is known. While the notion of a black hole originated from general relativity as a solution of the field equations, general relativity does not tell us how a black hole can be actually formed. A mechanism of black hole formation was provided by nuclear physics as a consequence of the gravitational collapse of a dead star. From our observations of the shape of massive objects , one would think the shape of a black hole is a sphere also. Since we don’t know (I mean I don’t know) for sure, it would be interesting to ponder whether the shape of a black hole different from a sphere can exist, for example a toroidal (doughnut shaped) black hole. If not for Schwarzschild black hole, how about for a rotational black hole?
Update: Now I know the answers to questions about the converse of Birkhoff’s theorem and the shape of a black hole. I wrote about them here. At the time of writing this article I knew even lesser than what I know now about GR, which is still pretty much close to nothing.
The Event Horizon
I conclude this article by mentioning that the Schwarzschild solution in \eqref{eq:bh} only describes the outside of the black hole. What determines the inside and the outside of a black hole? It is the event horizon. The event horizon is where $r=r_g=\frac{2MG}{c^2}$. $r_g$ is called the Schwarzschild radius.The Schwarzschild solution requires that $r>r_g$. The Schwarzschild solution unfortunately does not tell anything about whatever happens inside the black hole. We will never know for sure what happens once an object falls into a black hole past its event horizon unless we have information on the source of its gravity, i.e. the stress-energy tensor $T_{ij}$.